The history of DNA
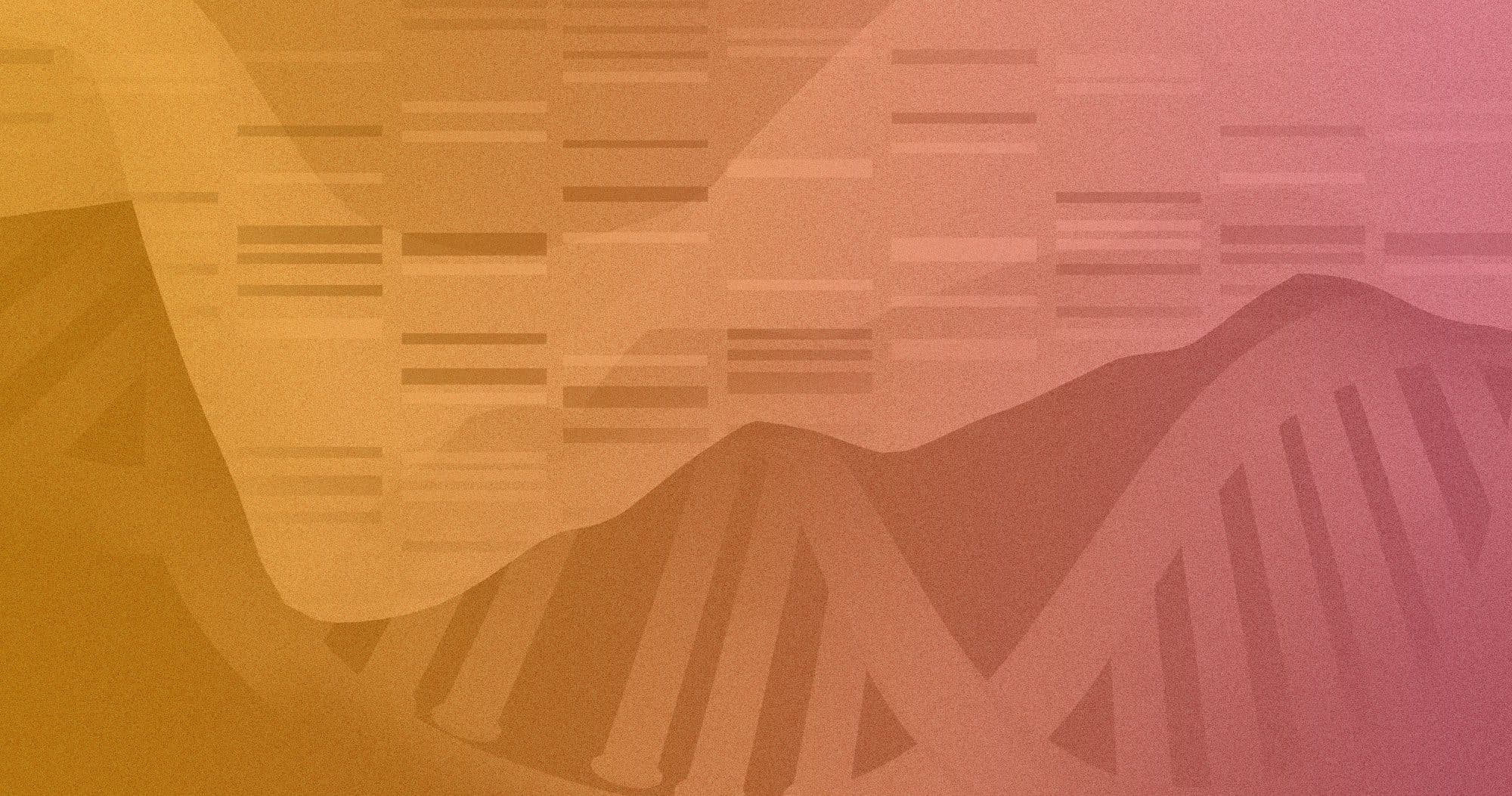
“If I have seen further, it is by standing on the shoulders of giants.”– Issac Newton
DNA is all around us. Not just in a physical sense, but in a cultural sense as well. We see it in our TV shows; we hear about the many ways our advanced understanding of it will revolutionize our lives. We even celebrate a National DNA Day.
DNA Day marks the anniversary of the publishing of Watson and Crick’s famous paper in Nature, describing the double helix structure of DNA. 2018 marks 60 years since that transformational event, and since that time, the world of genetics has boomed. It is now possible for anyone to have their DNA sequenced, the results of which can provide them with an ever-growing array of insights. But we haven’t always been able to use DNA like this. 150 years ago, people had no idea that DNA existed, and they certainly hadn’t heard of DNA sequencing. We’re able to do what we do thanks to the giants who came before us.
In honor of National DNA Day, we’ve assembled a list of some landmark events in the history of DNA. These events fed into one another, ultimately bringing us to our modern day understanding of DNA and genetics. So many people have been involved in this journey—leading to so many pivotal events in genetics—that it’s virtually impossible to mention them all in a single article. Instead, we’ve selected a few of the biggest moments.
Table of contents
1. 4 Billion Years Ago
2. 2.8 Million Years Ago
3. 1800 to 1900: The Birth of Genetics
4. 1900 to 1920: Understanding Traits
5. 1940s: Discovering the Nature of Genes
6. 1950s: A Decade of Titans
7. 1960s: Standing on the Shoulders of Giants
8. 1970 to 1990: Making Sense of the Sequence
9. 1990s to Today: The Human Genome Project and Beyond
A full history of DNA, of course, has to start at the beginning—the primordial soup of a very distant past. It’s believed that nearly 4 billion years ago, precursors to modern day DNA began to appear in this soup. There are competing theories about how exactly these precursors became DNA, but a common one states that nucleic acids formed in a series of reactions involving chemicals like cyanide, hydrochloric acid, and sugars. The nucleic acids began to assemble as RNA. Over time, DNA nucleotides also formed and incorporated themselves into the RNA structures, which then gave way to strands consisting solely of DNA nucleotides. Eventually, these evolved into a double helix—thus forming DNA as we know it.1,2,3.
Fast forward billions of years—not quite to modern day, but close, relatively speaking. At this point, DNA has come a long way since it first formed. It’s now packaged in membrane-bound cells, and has assembled into a code that gives rise to life. But evolution is a constant and continual process. Approximately 2.8 million years ago, the DNA of some bipedal apes evolved away from the others and generated the genus Homo. From this branch of life will grow Homo neanderthalensis, Homo denisova, and Homo sapiens—us4.



In the 19th century, humans began to make important scientific discoveries about cells and heredity
In the 19th century, humans began to make important scientific discoveries about cells and heredity. Farmers had actually already explored concepts in genetics by this point—unknowingly—through selective breeding of plants and animals. But the research done by a French zoologist, an English botanist, and an Augustinian monk would kick off an era of great advancement. In the early 1800s, Frenchman Jean Baptiste Lamarck began to publicly discuss his ideas about heredity (though he didn’t use that term). His lectures and writings would be the first concrete suggestions in modern science that life evolves and adapts as a result of environmental influence5. These ideas would be fleshed out and molded to more accurately describe life by botanist Charles Darwin in his seminal book On the Origin of Species, first published in 18595,6. Together, these two scientists would have a major impact on how we view life, evolution, and eventually DNA.
Around this same time, an Augustinian monk named Gregor Mendel was making discoveries that would later influence the entire field of genetics and how we interpret genetic tests—and he did it using pea plants. His work revealed that some traits are inherited in specific and predictable patterns, and that some traits are dominant, while others are recessive. The concepts revealed in his research helps modern day scientists understand patterns of inheritance and how our traits may be influenced by genes passed down from previous generations7. Mendel’s research was so important to the field that an entire type of genetic inheritance, Mendelian inheritance, is named after him.
During the 19th century, scientists around the world were beginning to describe biological factors that could be passed on from generation to generation which would affect our traits, although they weren’t sure what those factors might be. But in the early 20th century, scientists landed on the concept of a “gene.” As with Mendel, Wilhelm Johannsen was an influential scientist whose landmark studies were performed using plants (specifically bean plants, in his case). Johannsen showed that a bean’s weight was determined by genes it inherited from its parents, not from the population at large. In the course of his work, he coined the term gene to describe some unknown factor within cells that partially dictated an organism’s traits. Over time, as scientists learned more, the definition of a gene would evolve to include DNA. In the course of Johannsen’s writings, he also used terms like genotype to describe the features coming from genes, and phenotype as the traits we can see8—terms that we still use to this day.
In 1836, Heinrich Wilhelm Gottfried von Waldeyer-Hartz coined the term chromosome while examining cells under a microscope9. Nearly a century later, a handful of scientists began to connect the dots between genes and chromosomes. Nettie Stevens discovered that an organism’s biological sex was determined by its X chromosome in 190510. Shortly after that, Thomas Hunt Morgan proposed that chromosomes carried discrete units—genes—that are capable of determining an organism’s traits11. Over the next few decades, researchers would continue to describe the connection between heritable traits, disease, and their potential link to genes.
If the genetic discoveries made in the early 1900s represented a scientific spark, you could say that the ‘40s and ‘50s were a raging fire. Researchers continued to explore the concept of a “gene,” and the latest research had indicated that genes were discrete objects arranged in a fixed, linear fashion on chromosomes. No one imagined that genes could be capable of moving around; there didn’t seem to be a mechanism for this to happen, nor was there a known reason for them to need to move around. But Dr. Barbara McClintock proved otherwise.
Dr. McClintock used maize—commonly known as corn—in her studies. By examining chromosomes under a microscope and studying the inheritance patterns of corn traits like leaf and kernel color patterns, she showed that genes could move to different places in the genome. She called these segments of DNA transposable elements. It took decades before other geneticists accepted her work, but we know now that this phenomenon can be found throughout the kingdoms of life. Beyond simply observing transposable elements, she also reported that genes can control the activity of other genes by observing that a certain gene activated another depending on its location. She also observed that a transposable element could jump into the middle of another gene, causing it to function differently (or not at all).



No one imagined that genes could be capable of moving around
Up to this point in history, scientists had struggled to understand how genes actually had an influence on organisms. Dr. McClintock and others had described the relationship between genes, chromosomes, and our traits. Despite these early advances, they really didn’t understand how genes physically exerted their influence at the cellular level. Part of this was that scientists lacked an appropriate experimental model to study the phenomena. Though it had become common practice to use flies and maize in genetic studies, neither organism was particularly well suited for this kind of question. What they needed was a fast-growing, genetically straightforward organism with easily identifiable genetic traits. With this kind of model, researchers could have sufficient control over the experiment to ask how a genetic trait affects our biochemistry. Researchers found such a model in the fungus Neurospora crassa13.
Drs. George Beadle and Edward Tatum had previously studied genetics using a combination of methods. But in joining forces, they wanted to do something new. Rather than identifying heritable traits, they aimed to identify how genes were responsible for a specific biochemical process. N. crassa was perfect for this kind of question. Scientists just have to put a nutritious jelly—agar—into a petri dish, and then spread the fungal spores onto it. Researchers can add other materials to the agar which the fungus uses to build important biochemicals, without which it would die. Beadle and Tatum recognized that this process was stepwise: Material A was used to produce B, which was then used to produce C, and then C turned to D. This A–>B–>C–>D process was predictable, and perfect for determining if genes contributed to the process. If a gene is responsible for converting A into B, then breaking that gene should prevent a fungus from successfully converting material A into B. Through careful experimentation, Beadle and Tatum showed that genes affect an organism’s traits by influencing proteins, such as the protein that converts A to B. Their findings were revolutionary because geneticists not only learned how genes work, but they also gained an experimental process for studying the phenomena13.
It’s difficult to overstate the importance of the scientific breakthroughs of the 1950s. It was a non-stop stream of discovery that ultimately answered a question that had dogged geneticists for more than a century: What is the genetic material?
Chromosomes are biological structures made from a combination of DNA and proteins. Geneticists at the time understood that something within chromosomes held genetic information which could be passed down from generation to generation. But what was that material—the DNA or the proteins?
Answering this question took the efforts of many scientists over the span of a few decades. In the 1920s, Dr. Fredrick Griffith was studying two versions of the bacteria known as Streptococcus pneumoniae. He found that the pathogenic properties of one bacterial strain could be passed to the other, representing a form of genetic transfer in bacteria. Later, Oswald Avery, C. M. MacLeod, and M. McCarty would use these same bacteria to ask if that genetic material was made of proteins or DNA. They isolated the individual parts of debris from dead, pathogenic bacteria, which included DNA and proteins. They then bathed non-pathogenic bacteria in each individual component and found that only DNA was capable of transferring the pathogenic trait. This was the first definitive demonstration that DNA is the genetic material14.
The Avery, MaCleod, and McCarty experiments were well planned, but there was still a divide in the scientific community. Finally, in 1952, the argument was cemented by Martha Chase and Alfred Hershey. These researchers studied bacteriophages, viruses that infect bacteria by landing on a bacterium and injecting it with genetic material. That material is then used by the bacteria to build more viruses. Chase and Hershey radiolabeled the DNA of the bacteriophage to show that it’s only the DNA that gets passed on in the process of infection. Collectively, the Hershey-Chase and Avery, MaCleod, and McCarty experiments turned the tide in science and convinced many that DNA, and not protein, was the mystery genetic material scientists had talked about for so many years14.

She managed to take the first picture of DNA in its helical structure
Another significant blow would come from teams of researchers who were working to describe the structure of DNA. DNA is too small to be seen with the naked eye, even under a powerful microscope. Scientists had turned to a technique known as x-ray crystallography, which traps molecules in a crystal which is then blasted with x-rays. These x-rays bounce off of the trapped molecules in a unique pattern that allows researchers to determine the shape of the molecules in the crystal. Dr. Rosalind Franklin was an expert in the technique. She managed to take the first picture of DNA in its helical structure, but hadn’t yet worked out exactly what she was seeing.
Meanwhile, Dr. James Watson and Francis Crick had gained access to her research (a fact that remains controversial to this day, because the work was shared with them without Franklin’s knowledge). They recognized that the pattern shown in her image suggested a double helix. Using this information and other work describing that DNA had equal proportions of the complementary bases (equal numbers of A’s and T’s, and equal amounts of G’s and C’s), they assembled a model of the DNA structure and wrote the seminal paper describing the DNA double helix15. In this work, Watson and Crick indicated that DNA may function as a genetic material by coding information in the series of nucleotide bases and that it could be copied reliably based on the complementary nature of the double-stranded structure.
These researchers showed the world that DNA is a double helix, that it carries the genetic information passed from parent to child, and demonstrated the molecular mechanisms used by cells to build new strands of DNA16-18. Later, these findings would help us understand how variations in the DNA develop, how they’re propagated throughout time, and how this can lead to disease. The impact of this work truly makes these figures—Franklin, Chase, Hershey, and the rest—titans in the world of science.
Following the discovery of DNA as the genetic material, there was a strong drive to understand how information stored within the DNA could be used to build proteins. Ultimately, this effort resulted in the discovery of messenger RNA (mRNA). mRNA is a short lived molecule that is generated based on the DNA sequence of a gene. In humans, the mRNA is sent out of the nucleus to be used as a template for building proteins.
We know all of this thanks to the collective effort of numerous scientists in the 1940s and ‘50s. In the course of studying DNA, several observations were made which suggested the existence of mRNA. In the 1940s, experiments by Avery using bacteriophages noted the existence of a short lived RNA molecule that was made in large abundance following viral infection of a bacteria cell. While describing the structure of DNA, James Watson speculated about the existence of some mechanism that transported information in the DNA to the cytoplasm. The race to describe this mechanism intensified in the late 1950s, culminating in the publication of back-to-back papers describing the concept of mRNA in 1961. With this revelation, scientists now had a clearer picture of how DNA acted as the genetic material and how it was capable of influencing cell function19.
Breaking the genetic code
The next step to understanding DNA required, in a sense, code breakers. DNA is a series of four nucleotide bases—adenine, guanine, cytosine, and thymine. Somehow, these four bases are arranged in a certain way—a code—that makes them capable of giving instructions for how to build proteins. In the early 1960s, that code was broken by the combined efforts of separate research teams lead by Robert W. Holley, H. Gobind Khorana, and Marshall W. Nirenberg.
The 1950s had showed that DNA carried genetic material, and there was mounting evidence suggesting that RNA was used to build proteins. It was thought that the sequence of DNA determined the sequence of RNA, and in turn RNA would determine the sequence of amino acids used to build a protein. However, it was unclear how the sequence of RNA directed the addition of a specific amino acid. There were four possible nucleotide bases in RNA, but 20 possible amino acids. This indicated that multiple bases, arranged in the right order, coded for a specific amino acid.
Geneticists anticipated that it would take decades to decipher the exact number and order of this genetic code, but Dr. Nirenberg had a plan. He wanted to synthetically create RNA molecules consisting of only one letter (uracil, U), repeated over and over again. He would then combine this with ribosomes—the machinery responsible for making proteins—in a test tube. The final step was to add in one of the 20 amino acids that could be used to build proteins. He did this for each amino acid until one of them generated a protein. At that point, he had to slightly alter the RNA sequence to determine what the genetic code was—a seemingly small task that took years and the work of multiple labs.
We now know that specific amino acids are added to a protein based on unique sequences of 3 nucleotides in the RNA. Scientists refer to these sequences as codons. In 1961, Dr. Nirenberg published his initial findings and showed the world its first real look at a codon: the repeated sequence of UUU resulted in a long string of a single amino acid known as phenylalanine. Over the next few years, Dr. Nirenberg, Dr. Khorana, and Dr. Holley separately contributed to deciphering the exact correlation between codons and their corresponding amino acids. For their work, the trio shared in the 1968 Nobel prize for Physiology and Medicine20-22.
At this point, scientists now had a key to help them translate the language of DNA. However, there was still a significant problem ahead of them: they had no practical way to determine the DNA sequence of an organism. Functionally, they were able to see what letters were present in a genome, but they couldn’t determine the order of those letters. If humanity was to go further in its study of DNA, a technological revolution was needed.


A technological revolution was needed
In the early days of DNA sequencing, researchers focused much of their effort on RNA because its structure is a bit simpler. Unlike DNA, RNA is single-stranded and could be produced in large quantities by viruses and microorganisms. Robert Holley and his colleagues successfully sequenced the full nucleic acid sequence of a tRNA (specifically the one coding for the amino acid alanine) in 1965. tRNA is used by a cell when building proteins—it’s what connects specific amino acids to specific mRNA sequences. Dr. Holley’s discovery significantly helped the ongoing efforts to characterize codons. Simultaneously, Dr. Fred Sanger and others were developing a new method of sequencing that would allow them to take lessons from RNA sequencing and apply them to DNA23.
In 1972, a team of researchers led by Dr. Walter Fiers used a modified version of Sanger’s sequencing method to generate the complete DNA sequence of a bacteriophage protein, making it the first complete DNA sequence of a protein coding gene. In the years that followed, DNA sequencing technology rapidly evolved to become more efficient and more accurate. Then in 1977, a powerful sequencing method was developed which we now know as Sanger sequencing.
Due to the simplicity of Sanger sequencing, the technique spread through the science community rapidly. Over time, technology used for this method would evolve to enable automation of the sequencing process and make it possible to sequence as many as 1,000 bases in a single run—a tiny fraction of the billions of bases in the human genome, but an incredible breakthrough for its time. And ultimately, Sanger sequencing would give rise to the technology used to sequence the human genome, inspiring the next generation sequencing (NGS) technology used in many modern sequencing labs23. NGS reads millions of small DNA fragments, multiple times, to give scientists an accurate picture of what the DNA sequence looks like and allows them to identify small changes in a person’s DNA sequence with confidence.
The 1980s gave rise to another critical sequencing technology—a method known as polymerase chain reaction (PCR), which was developed by Dr. Kary Mullis and significantly improved the potential that sequencing held. A major hurdle in DNA sequencing was that they needed large amounts of DNA, which was difficult to come by. PCR enabled researchers to take a small amount of DNA and turn it into a much larger amount which could then be sequenced.
Splicing RNA
Compared to a DNA sequence of a gene, its corresponding mRNA is typically shorter. mRNA is a single-stranded molecule that is made according to the DNA sequence of a gene, so if it is based on the DNA sequence, how could it be shorter? In the late 1970s, two different researchers would come to the same conclusion: portions of a gene sequence are removed from the mRNA before it is sent to direct protein synthesis.
In the process of making mRNA, your body first makes pre-mRNA. This molecule is almost an exact replica of the DNA sequence it was made from. Researchers working under Dr. Phillip Sharp—and, separately, Dr. Richard J. Roberts—found that segments of the pre-mRNA molecule did not correspond to the amino acid sequence of the final protein and that they appeared to be removed as soon as the pre-mRNA was formed. The segments of the pre-mRNA that were removed were called introns. Once the introns were removed and a few more modifications took place, the mRNA was formed. The sequences that remained in the mRNA were called exons, and it is the exons that actually code for the final protein product24.
Removing introns and then gluing the exons together is a process known as splicing, and its discovery radically affected how we view genetics. The fact that protein coding RNA is broken up into pieces and then parts of it were glued together again showed a level of complexity that we hadn’t expected. In fact, during this process, sometimes genes change which parts of the pre-mRNA they cut out, and sometimes shuffle the order of the exons. This can have a dramatic effect on the shape and function of the resulting protein. We now known this process as alternative splicing and exon shuffling25.
By the 1990s, the significant advances in DNA sequencing technology from the previous decades had prompted scientists to consider a new, radical project. This project would aim to sequence the entire human genome and establish a baseline human DNA sequence. If researchers could do this, it would allow them to understand where a person’s DNA may differ from the baseline, and then study how that difference may have affected them. It would also allow us to better characterize the information coded in our genes—and even the number of genes we have in the first place. This radical project, the Human Genome Project, officially began in 1990.
In 2003, nearly 50 years to the day after the publication of Watson and Crick’s historic paper, the Human Genome Project published the first-ever complete sequence of the human genome. This monumental accomplishment signaled the increasing power of DNA sequencing technology. When the project began, scientists estimated that it would take 15 years to complete. Instead, the rapid pace of innovation had enabled the project to develop new sequencing technologies and speed ahead of projections.
With these new technologies, and with the full sequence of the human genome freely available to researchers, the stage was set for a new wave of discoveries about the human genome. Since the project ended, we’ve learned that the human genome consists of more than 20,000 different genes, and we’ve learned that these genes have evolved over time. Continuing innovations to sequencing technology have vastly improved our ability to study DNA and characterize its influence on us. In the process, we’ve gained more and more insights into how mutations precipitated the evolution of all life on earth.

The power of DNA sequencing is no longer limited to the academic setting
But the power of DNA sequencing is no longer limited to the academic setting. The first generation of consumer genetics companies and projects began appearing in the first years after the Human Genome Project ended. For example, in 2005, our partner National Geographic launched the Genographic Project, which aimed to analyze the DNA of thousands of people around the world—particularly people from indigenous communities—in order to build a more complete picture of ancient human migration patterns. Along with other early consumer genetics efforts (and the Genographic consumer products that National Geographic has launched since), the Genographic Project helped bring DNA sequencing to the masses.
More than a century of research has illuminated the power of DNA. The efforts of thousands of researchers have collectively led us to where we are in genomic research and technology today, and the numerous ways in which the power of the genome can impact our lives. It was only 100 years ago that scientists were learning about chromosomes. Now, anyone can have their DNA sequenced to gain insights. Inspired by the giants that came before us, Helix is taking another step by sequencing your entire exome—the regions of the DNA that code for proteins—and other areas of interest within the genome. With this information, you can gain insights about your distant ancestry, or you can learn about your DNA and the ways it could be influencing your body’s response to various foods. You could use this information to ask if you’ve inherited a variant in the DNA that predisposes you to a serious disease, or you can use your DNA to inspire art. The possibilities are endless, and we’re witnessing that with the exciting new products and partners that are launching all the time.
We’ve come a long way since the days of Darwin and Mendel. While we still have a long way to go before we fully understand the human genome, it’s incredible to look at how much has changed—and it’s easy to get excited about what the future may hold.
1Wächtershäuser, Günter. “The Place of RNA in the Origin and Early Evolution of the Genetic Machinery.” Ed. Niles Lehman. Life 4.4 (2014): 1050–1091. PMC. Web. 12 Apr. 2018.
2Alberts B, Johnson A, Lewis J, et al. Molecular Biology of the Cell. 4th edition. New York: Garland Science; 2002. The RNA World and the Origins of Life. Available from: https://www.ncbi.nlm.nih.gov/books/NBK26876/
3E., Orgel Leslie. “Prebiotic Chemistry and the Origin of the RNA World.” Critical Reviews in Biochemistry and Molecular Biology, vol. 39, no. 2, 2004, pp. 99–123., doi:10.1080/10409230490460765.
4Foley, Robert A. et al. “Major Transitions in Human Evolution.” Philosophical Transactions of the Royal Society B: Biological Sciences 371.1698 (2016): 20150229. PMC. Web. 12 Apr. 2018.
5Burkhardt, Richard W. “Lamarck, Evolution, and the Inheritance of Acquired Characters.” Genetics 194.4 (2013): 793–805. PMC. Web. 12 Apr. 2018.
6”Rewriting the Book of Nature – Charles Darwin and Evolutionary Theory.” U.S. National Library of Medicine, National Institutes of Health, 29 July 2013, www.nlm.nih.gov/exhibition/darwin/evolutionarytheory.html.
7De Castro, Mauricio. “Johann Gregor Mendel: Paragon of Experimental Science.” Molecular Genetics & Genomic Medicine 4.1 (2016): 3–8. PMC. Web. 12 Apr. 2018.
8Roll-Hansen, Nils. “The Holist Tradition in Twentieth Century Genetics. Wilhelm Johannsen’s Genotype Concept.” The Journal of Physiology 592.Pt 11 (2014): 2431–2438. PMC. Web. 12 Apr. 2018.
9Roll-Hansen, Nils. “The Holist Tradition in Twentieth Century Genetics. Wilhelm Johannsen’s Genotype Concept.” The Journal of Physiology 592.Pt 11 (2014): 2431–2438. PMC. Web. 12 Apr. 2018.
10Wessel, Gary M. “Y Does It Work This Way? Nettie Maria Stevens (July 7, 1861 – May 4, 1912).” Molecular Reproduction and Development, vol. 78, no. 9, 2011, doi:10.1002/mrd.21390.
11Griffiths, Anthony JF. “Historical Development of the Chromosome Theory.” An Introduction to Genetic Analysis. 7th Edition., U.S. National Library of Medicine, 1 Jan. 1970, www.ncbi.nlm.nih.gov/books/NBK22088/.
12Ravindran, Sandeep. “Barbara McClintock and the Discovery of Jumping Genes.” Proceedings of the National Academy of Sciences of the United States of America 109.50 (2012): 20198–20199. PMC. Web. 23 Apr. 2018.
13Strauss, Bernard S. “Biochemical Genetics and Molecular Biology: The Contributions of George Beadle and Edward Tatum.” Genetics 203.1 (2016): 13–20. PMC. Web. 23 Apr. 2018.
14Griffiths, Anthony JF. “DNA: The Genetic Material.” An Introduction to Genetic Analysis. 7th Edition., U.S. National Library of Medicine, 1 Jan. 1970, www.ncbi.nlm.nih.gov/books/NBK22104/.
15“The Rosalind Franklin Papers: The DNA Riddle: King’s College, London, 1951-1953.” U.S. National Library of Medicine, National Institutes of Health, profiles.nlm.nih.gov/ps/retrieve/Narrative/KR/p-nid/187.
16“The Arthur Kornberg Papers: The Synthesis of DNA, 1953-1959.” U.S. National Library of Medicine, National Institutes of Health, profiles.nlm.nih.gov/ps/retrieve/Narrative/WH/p-nid/208.
17Tan, Siang Yong, and Kate Pettigrew. “Severo Ochoa (1905–1993): The Man behind RNA.” Singapore Medical Journal 59.1 (2018): 3–4. PMC. Web. 23 Apr. 2018.
18Hanawalt, Philip C. “Density Matters: The Semiconservative Replication of DNA.” Proceedings of the National Academy of Sciences of the United States of America 101.52 (2004): 17889–17894. PMC. Web. 23 Apr. 2018.
19Cobb, Matthew. “Who Discovered Messenger RNA?” Current Biology, vol. 25, no. 13, 2015, doi:10.1016/j.cub.2015.05.032.
20“Nirenberg: History Section: Cracked Code.” National Institutes of Health, U.S. Department of Health and Human Services, history.nih.gov/exhibits/nirenberg/HS5_cracked.htm.
21“Nirenberg: History Section: Poly-U.” National Institutes of Health, U.S. Department of Health and Human Services, history.nih.gov/exhibits/nirenberg/HS4_polyU.htm.
22Marshall, Jessica. “The Genetic Code.” Proceedings of the National Academy of Sciences of the United States of America 111.16 (2014): 5760. PMC. Web. 24 Apr. 2018.
23Heather, James M., and Benjamin Chain. “The Sequence of Sequencers: The History of Sequencing DNA.” Genomics 107.1 (2016): 1–8. PMC. Web. 24 Apr. 2018.
24Berk, Arnold J. “Discovery of RNA Splicing and Genes in Pieces.” Proceedings of the National Academy of Sciences of the United States of America 113.4 (2016): 801–805. PMC. Web. 24 Apr. 2018.
25Gilbert, Scott F. “Differential RNA Processing.” Developmental Biology. 6th Edition., U.S. National Library of Medicine, 1 Jan. 1970, www.ncbi.nlm.nih.gov/books/NBK10112/.
Categories